Interview: The truth about our universe
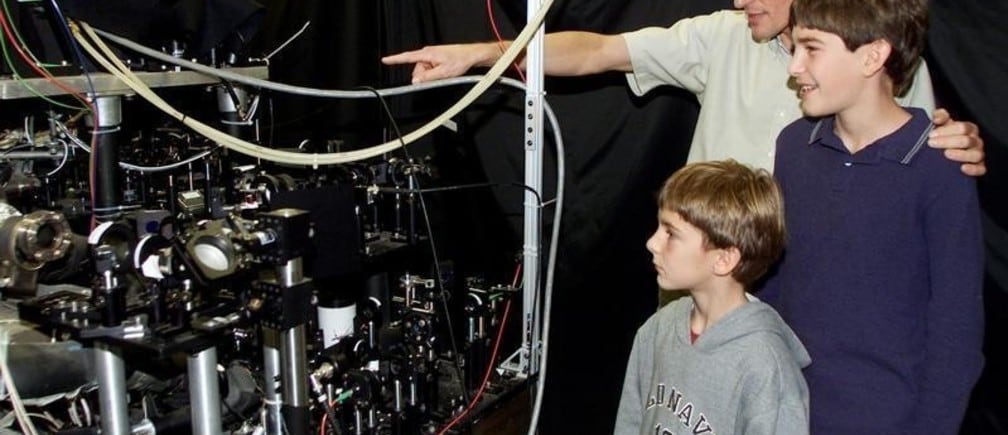
Guest editor Rafael Reif, President of MIT, writes: “This interview with MIT physicist (and Nobel laureate) Wolfgang Ketterle is very interesting reading about the high value of basic, curiosity-driven research. Without it, as Wolfgang says, ‘we will have no new game-changing technologies for tomorrow’s applied research.'”
Your lab cools matter to extremely low temperatures – just above absolute zero (minus 273.15°C). Why?
Matter changes in interesting ways at ultra-cold temperatures. As an analogy, imagine you lived in a tropical climate and had never before encountered a refrigerator. As you watched water cool, at first you would see that nothing much changes – then the temperature reaches a certain point and something completely new and very interesting happens. The water becomes solid, it becomes ice.
Most people are used to thinking of the basic states of matter as being solid, liquid or gas. However, those are not the only possible states. At extremely low temperatures, matter can enter an entirely different state, called a superfluid.
This is the Bose-Einstein Condensate, for which you won your Nobel Prize.
Bose and Einstein developed equations which predicted that, in theory, if you could get matter cold enough, then the atoms would start to behave in the same way and act as a single wave. Imagine a busy city street, with people bustling around and getting in each other’s way, and then imagine that all those people suddenly start to march in lockstep. Something like this is what happens.
Einstein remarked that the equations were elegant, but it was impossible to know if the states of matter they predicted were possible in practice. Once we had proved it was possible to create a Bose-Einstein Condensate in the lab, it opened up a whole new area of fundamental research.
How do you create a superfluid in the lab?
Bose-Einstein condensates need to be created directly from gas, so first we have to prevent the gas from going through the liquid and solid phases. We do this by creating a gas that is a billion times more dilute – meaning the atoms are spaced a thousand times further away from each other, on each of the three dimensions, to prevent them from bonding. Since the atoms are so spaced out, we are talking about only microscopic quantities of such gases.
Then we use a variety of techniques to manipulate those atoms down in temperature, such as lasers, microwaves and radio waves. It’s a physical impossibility to reach absolute zero, but we can get to within hundreds of trillionths of a degree, which is cold enough to do our work.
Once we have matter in an ultra-cold state, we manipulate it and try to construct new things with it. You can think of our work as being rather like a child’s games of Lego, only our Lego blocks are atoms and we are moving them around using laser beams. Just as a child uses Lego blocks to experiment with creating new things, so we are seeing what it is and isn’t possible to construct in nature. Effectively we are creating designer matter, which is unprecedented.
And what do you hope to learn?
First and foremost it is deeply exciting to map what is physically possible. What we are creating in the lab are forms of matter that have never before been observed. There are no new places left in the world for explorers to discover, and it’s very hard to discover a new species of animal, but in science we are discovering new things all the time.
In a practical sense, ultimately the hope is that if we understand how to manipulate small amounts of matter at very low temperatures, then we can figure out how to scale it up and get that same behaviour at more normal temperatures.
What are examples of properties matter displays at very low temperatures, and are there already real-world examples where that behaviour is replicated at higher temperatures?
One of the properties of matter at very low temperatures is superconductivity, and it is already possible to achieve superconductivity up to the boiling point of liquid nitrogen – still very cold, of course, but a much higher temperature than absolute zero. Real world applications of superconductivity include powerful magnets for MRI scanners, particle accelerators and MAGLEV (magnetic levitation) trains.
If we could discover how to achieve superconductivity at room temperatures, the implications would be revolutionary. For example, we could eliminate efficiency losses in electricity distribution grids, and enable much more powerful motors and microchips as you would no longer have to contend with the heat currently generated as a by-product of conducting energy.
Another area of our research is to understand magnetic materials and phase transitions in general.
How likely is it that you will figure out superconductivity at room temperature?
There is no fundamental law of physics that says it isn’t possible. And if it isn’t theoretically impossible, then we have to try to achieve it.
Of course, it is impossible to estimate when it might happen, because this is fundamental research. You can’t predict when somebody will make a disruptive discovery, or have a breakthrough idea.
As nobody can tell what practical applications might follow from your work, or on what timescale, presumably your research relies on public funding?
Yes. Effectively, my lab is pretty much funded by the taxpayer, via the National Science Foundation and the Department of Defence.
In these difficult economic times, do you feel there is enough appreciation of the need to invest in fundamental research?
It is certainly more difficult to do fundamental research than it was even a decade or two ago – let alone in the era of Sputnik and the Apollo missions, when fundamental research was a high priority – and I speak as someone who is in a relatively privileged position. There has been more emphasis in recent times on funding applied research, which aims to solve a specific practical problem, rather than fundamental research, which is driven by curiosity.
We need both kinds of research, as without fundamental research today we will have no new game-changing technologies for tomorrow’s applied research. Dentists, for example, use lasers, which were invented by people who were researching light. If lasers didn’t yet exist, no amount of research in dentistry would ever be likely to invent them.
Or think of the enormous social and economic value being created by GPS, which depends on accurate timekeeping made possible by atomic clocks – an area of research close to my own. Those atomic researchers would never have anticipated that their discoveries would end up making possible location-based Internet searches or driverless vehicles.
You can say two things about investing in fundamental research. You don’t know how it will pay off. But you can be absolutely confident that it will pay off, handsomely, because in one way or another it always has.
You’re confident that the work you’re doing now will inform technologies that will change our lives in the coming decades?
If you ask me to predict the future, all I can do is extrapolate from the past – and the rates of discovery in this field have been rapid and accelerating. There is an enormous amount of excitement.
So, yes. Although I can’t predict what they will be, I am confident that the insights we are obtaining today will lead, in 10 or 20 or 30 years, to novel materials that will have far-reaching implications.
Wolfgang Ketterle is professor of physics at the Massachusetts Institute of Technology (MIT). He is participating in the World Economic Forum Annual Meeting 2014 in Davos.
Image: Dr. Wolfgang Ketterle, winner of the 2001 Nobel Prize for Physics shows the first generation Bose-Einstein sodium condensate machine that he began his research on. REUTERS/Jim Bourg
Don't miss any update on this topic
Create a free account and access your personalized content collection with our latest publications and analyses.
License and Republishing
World Economic Forum articles may be republished in accordance with the Creative Commons Attribution-NonCommercial-NoDerivatives 4.0 International Public License, and in accordance with our Terms of Use.
The views expressed in this article are those of the author alone and not the World Economic Forum.
Forum Stories newsletter
Bringing you weekly curated insights and analysis on the global issues that matter.